The Dose-Dependent Role of Sage
The Dose-Dependent Role of Sage, Clove, and Pine Essential Oils in Modulating Ruminal Fermentation and Biohydrogenation of Polyunsaturated Fatty Acids: A Promising Strategy to Reduce Methane Emissions and Enhance the Nutritional Profile of Ruminant Products
The Dose-Dependent Role of Sage, Clove, and Pine Essential Oils in Modulating Ruminal Fermentation and Biohydrogenation of Polyunsaturated Fatty Acids: A Promising Strategy to Reduce Methane Emissions and Enhance the Nutritional Profile of Ruminant Products
Featured Application
Abstract
1. Introduction
2. Materials and Methods
2.1. Diet, Donor Animals, and Microbial Inoculum
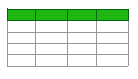
2.2. Plant Material and Preparation
2.3. Extraction of Essential Oils
2.4. Essential Oil Analysis
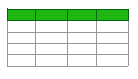
2.5. Experimental Design
2.6. Gas Production and Rumen Fermentation Kinetics
The measured gas production data were processed to fit with the model proposed by France et al. [28] as follows:
where GP is the gas production, B is the ideal maximum gas production, C is the gas production rate, T is time of incubation, and L is lag time prior to gas production commencing. The parameters for the kinetics of rumen fermentation (B, C, and L) were estimated using the nonlinear regression procedure of Statistical Analysis Software version 9.4 (SAS 9.4; SAS Institute, Inc., Cary, NC, USA). Subsequently, the time taken to reach half of the ideal maximum GP (HT) and the average GP rate (AGPR) at HT was calculated using the following equations according to Kong et al. [29]:
2.7. Determination of Methane and Carbon Dioxide
2.8. Sampling, and Measurements
2.9. Chemical Analysis
2.10. Data analysis
3. Results
3.1. Gas Production and Kinetics of Ruminal Fermentation
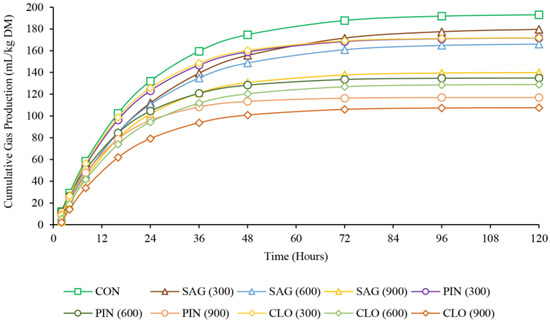
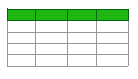
3.2. Production of Methane and Carbon Dioxide
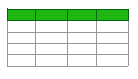
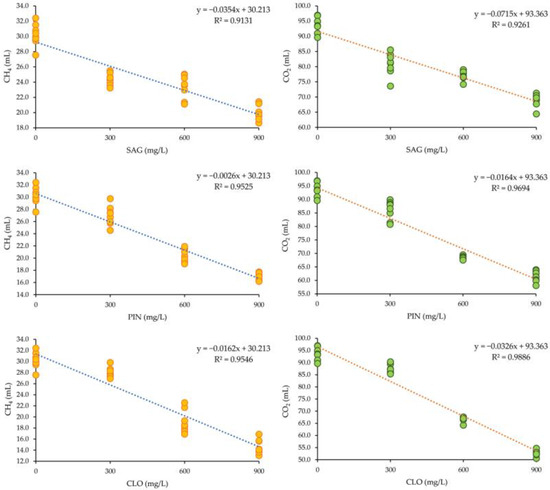
3.3. In Vitro Ruminal Fermentation
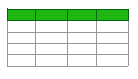
3.4. In Vitro Concentrations of Fatty Acids
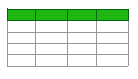
3.5. In Vitro Biohydrogenation of Ruminal PUFA
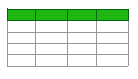
4. Discussion
4.1. Gas Production and Kinetics of Ruminal Fermentation
4.2. Kinetics of Rumen Fermentation
4.3. Production of Methane and Carbon Dioxide
4.4. In Vitro Ruminal Fermentation
4.5. In Vitro Biohydrogenation and Fatty Acid Concentrations
5. Conclusions
Author Contributions
Funding
Institutional Review Board Statement
Informed Consent Statement
Data Availability Statement
Acknowledgments
Conflicts of Interest
References
- Gerber, P.J.; Steinfeld, H.; Henderson, B.; Mottet, A.; Opio, C.; Dijkman, J.; Falcucci, A.; Tempio, G. Tackling Climate Change through Livestock: A Global Assessment of Emissions and Mitigation Opportunities; Food and Agriculture Organization of the United Nations (FAO): Rome, Italy, 2013. [Google Scholar]
- Monteiro, V.C.; Miles, N.L.; Richardson, S.J.; Barkley, Z.; Haupt, B.J.; Lyon, D.; Hmiel, B.; Davis, K.J. Methane, carbon dioxide, hydrogen sulfide, and isotopic ratios of methane observations from the Permian Basin tower network. Earth Syst. Sci. Data 2022, 14, 2401–2417. [Google Scholar] [CrossRef]
- Zisis, F.; Kyriakaki, P.; Satolias, F.F.; Mavrommatis, A.; Simitzis, P.E.; Pappas, A.C.; Surai, P.F.; Tsiplakou, E. The Effect of Dietary Inclusion of Microalgae Schizochytrium spp. on Ewes’ Milk Quality and Oxidative Status. Foods 2022, 11, 2950. [Google Scholar] [CrossRef] [PubMed]
- Delgado-Pertíñez, M.; Horcada, A. Better Animal Feeding for Improving the Quality of Ruminant Meat and Dairy. Foods 2021, 10, 1076. [Google Scholar] [CrossRef]
- Correddu, F.; Caratzu, M.F.; Lunesu, M.F.; Carta, S.; Pulina, G.; Nudda, A. Grape, Pomegranate, Olive, and Tomato By-Products Fed to Dairy Ruminants Improve Milk Fatty Acid Profile without Depressing Milk Production. Foods 2023, 12, 865. [Google Scholar] [CrossRef] [PubMed]
- Bokharaeian, M.; Toghdory, A.; Ghoorchi, T.; Ghassemi Nejad, J.; Esfahani, I.J. Quantitative Associations between Season, Month, and Temperature-Humidity Index with Milk Yield, Composition, Somatic Cell Counts, and Microbial Load: A Comprehensive Study across Ten Dairy Farms over an Annual Cycle. Animals 2023, 13, 3205. [Google Scholar] [CrossRef]
- Nguyen, Q.V.; Malau-Aduli, B.; Cavalieri, J.; Malau-Aduli, A.E.O.; Nichols, P. Enhancing Omega-3 Long-Chain Polyunsaturated Fatty Acid Content of Dairy-Derived Foods for Human Consumption. Nutrients 2019, 11, 743. [Google Scholar] [CrossRef]
- Benetel, G.; Silva, T.D.S.; Fagundes, G.M.; Welter, K.C.; Melo, F.A.; Lobo, A.A.G.; Muir, J.P.; Bueno, I.C.S. Essential Oils as In vitro Ruminal Fermentation Manipulators to Mitigate Methane Emission by Beef Cattle Grazing Tropical Grasses. Molecules 2022, 27, 2227. [Google Scholar] [CrossRef]
- Fusaro, I.; Cavallini, D.; Giammarco, M.; Serio, A.; Mammi, L.M.E.; De Matos Vettori, J.; Lanzoni, L.; Formigoni, A.; Vignola, G. Effect of Diet and Essential Oils on the Fatty Acid Composition, Oxidative Stability and Microbiological Profile of Marchigiana Burgers. Antioxidants 2022, 11, 827. [Google Scholar] [CrossRef]
- Torres, R.N.S.; Moura, D.C.; Ghedini, C.P.; Ezequiel, J.M.B.; Almeida, M.T.C. Meta-analysis of the effects of essential oils on ruminal fermentation and performance of sheep. Small Rumin. Res. 2020, 189, 106148. [Google Scholar] [CrossRef]
- Sandner, G.; Heckmann, M.; Weghuber, J. Immunomodulatory Activities of Selected Essential Oils. Biomolecules 2020, 10, 1139. [Google Scholar] [CrossRef]
- Temiz, U.; Ozturk, E. Antioxidant and Immunostimulant Effects of Some Medicinal and Aromatic Plants. In Proceedings of the 4th International Eurasian Agriculture and Natural Sciences Congress, Online, 30–31 October 2020; pp. 312–317. Available online: https://online.agrieurasia.com/TR/ (accessed on 22 October 2023).
- Tsiplakou, E.; Pitino, R.; Manuelian, C.L.; Simoni, M.; Mitsiopoulou, C.; De Marchi, M.; Righi, F. Plant Feed Additives as Natural Alternatives to the Use of Synthetic Antioxidant Vitamins in Livestock Animal Products Yield, Quality, and Oxidative Status: A Review. Antioxidants 2021, 10, 780. [Google Scholar] [CrossRef] [PubMed]
- Kelly, L.; Kebreab, E. Recent advances in feed additives with the potential to mitigate enteric methane emissions from ruminant livestock. J. Soil Water Conserv. 2023, 78, 111–123. [Google Scholar] [CrossRef]
- Vasta, V.; Daghio, M.; Cappucci, A.; Buccioni, A.; Serra, A.; Viti, C.; Mele, M. Invited review: Plant polyphenols and rumen microbiota responsible for fatty acid biohydrogenation, fiber digestion, and methane emission: Experimental evidence and methodological approaches. J. Dairy Sci. 2019, 102, 3781–3804. [Google Scholar] [CrossRef]
- Dias, P.C.G., Jr.; dos Santos, I.J.; da Silva, A.L.A.; de Assis, R.G.; Vicente, A.C.S.; Carlis, M.S.P.; Soares, L.C.B.; Comelli, J.H.; Biava, J.S.; Araujo, R.C.; et al. Essential oil from Arnica montana on feedlot performance, ingestive behavior, carcass characteristics, rumen morphometrics characteristics and meat fatty acids profile of lambs. Small Rumin. Res. 2023, 220, 106920. [Google Scholar] [CrossRef]
- Daning, D.R.A.; Hanim, C.; Widyobroto, B.P.; Yusiati, L.M. Characteristics of Ruminal Fatty Acids Using In vitro Culture System by Addition of Galangal Alpinia galangal Essential oil. In Proceedings of the 9th International Seminar on Tropical Animal Production (ISTAP 2021), Yogyakarta, Indonesia, 21–22 September 2021. [Google Scholar]
- Coşkuntuna, L.; Lackner, M.; Erten, K.; Gül, S.; Palangi, V.; Koç, F.; Esen, S. Greenhouse Gas Emission Reduction Potential of Lavender Meal and Essential Oil for Dairy Cows. Fermentation 2023, 9, 253. [Google Scholar] [CrossRef]
- Elghandour, M.M.M.Y.; Maggiolino, A.; García, E.I.C.; Sánchez-Aparicio, P.; De Palo, P.; Ponce-Covarrubias, J.L.; Pliego, A.B.; Salem, A.Z.M. Effects of Microencapsulated Essential Oils on Equine Health: Nutrition, Metabolism and Methane Emission. Life 2023, 13, 455. [Google Scholar] [CrossRef]
- Bach, A.; Elcoso, G.; Escartín, M.; Spengler, K.; Jouve, A. Modulation of milking performance, methane emissions, and rumen microbiome on dairy cows by dietary supplementation of a blend of essential oils. Animal 2023, 17, 100825. [Google Scholar] [CrossRef]
- Kim, M.J.; Jung, U.S.; Jeon, S.W.; Lee, J.S.; Kim, W.S.; Lee, S.B.; Kim, Y.C.; Kim, B.Y.; Wang, T.; Lee, H.G. Improvement of Milk Fatty Acid Composition for Production of Functional Milk by Dietary Phytoncide Oil Extracted from Discarded Pine Nut Cones (Pinus koraiensis) in Holstein Dairy Cows. Asian-Australas. J. Anim. Sci. 2016, 29, 1734–1741. [Google Scholar] [CrossRef]
- Heidarian Miri, V.; Tyagi, A.K.; Ebrahimi, S.H.; Mohini, M. Effect of cumin (Cuminum cyminum) seed extract on milk fatty acid profile and methane emission in lactating goat. Small Rumin. Res. 2013, 113, 66–72. [Google Scholar] [CrossRef]
- Rana, M.S.; Tyagi, A.; Hossain, S.A.; Tyagi, A.K. Effect of tanniniferous Terminalia chebula extract on rumen biohydrogenation, ∆9-desaturase activity, CLA content and fatty acid composition in longissimus dorsi muscle of kids. Meat Sci. 2012, 90, 558–563. [Google Scholar] [CrossRef]
- Yáñez-Ruiz, D.R.; Bannink, A.; Dijkstra, J.; Kebreab, E.; Morgavi, D.P.; O’Kiely, P.; Reynolds, C.K.; Schwarm, A.; Shingfield, K.J.; Yu, Z.; et al. Design, implementation and interpretation of in vitro batch culture experiments to assess enteric methane mitigation in ruminants—A review. Anim. Feed Sci. Technol. 2016, 216, 1–18. [Google Scholar] [CrossRef]
- NRC. Nutrient Requirements of Small Ruminants: Sheep, Goats, Cervids, and New World Camelids; The National Academies Press: Washington, DC, USA, 2007; p. 384. [Google Scholar]
- Theodorou, M.K.; Williams, B.A.; Dhanoa, M.S.; McAllan, A.B.; France, J. A simple gas production method using a pressure transducer to determine the fermentation kinetics of ruminant feeds. Anim. Feed Sci. Technol. 1994, 48, 185–197. [Google Scholar] [CrossRef]
- Mauricio, R.M.; Mould, F.L.; Dhanoa, M.S.; Owen, E.; Channa, K.S.; Theodorou, M.K. A semi-automated in vitro gas production technique for ruminant feedstuff evaluation. Anim. Feed Sci. Technol. 1999, 79, 321–330. [Google Scholar] [CrossRef]
- France, J.; Dijkstra, J.; Dhanoa, M.S.; Lopez, S.; Bannink, A. Estimating the extent of degradation of ruminant feeds from a description of their gas production profiles observed in vitro: Derivation of models and other mathematical considerations. Br. J. Nutr. 2000, 83, 143–150. [Google Scholar] [CrossRef] [PubMed]
- Kong, F.; Wang, S.; Cao, Z.; Wang, Y.; Li, S.; Wang, W. In vitro Fermentation and Degradation Characteristics of Rosemary Extract in Total Mixed Ration of Lactating Dairy Cows. Fermentation 2022, 8, 461. [Google Scholar] [CrossRef]
- AOAC. Official Methods of Analysis of the Association of Official Analytical Chemists, 21st ed.; AOAC International: Washington, DC, USA, 2019. [Google Scholar]
- Mertens, D.R. Gravimetric determination of amylase-treated neutral detergent fiber in feeds with refluxing in beakers or crucibles: Collaborative study. J. AOAC Int. 2002, 85, 1217–1240. [Google Scholar]
- Weatherburn, M.W. Phenol-hypochlorite reaction for determination of ammonia. Anal. Chem. 1967, 39, 971–974. [Google Scholar] [CrossRef]
- Muck, R.E.; Dickerson, J.T. Storage Temperature Effects on Proteolysis in Alfalfa Silage. Trans. ASAE 1988, 31, 1005–1009. [Google Scholar] [CrossRef]
- Wachira, A.M.; Sinclair, L.A.; Wilkinson, R.G.; Hallett, K.; Enser, M.; Wood, J.D. Rumen biohydrogenation of n-3 polyunsaturated fatty acids and their effects on microbial efficiency and nutrient digestibility in sheep. J. Agric. Sci. 2000, 135, 419–428. [Google Scholar] [CrossRef]
- Vera, N.; Gutiérrez-Gómez, C.; Williams, P.; Allende, R.; Fuentealba, C.; Ávila-Stagno, J. Comparing the Effects of a Pine (Pinus radiata D. Don) Bark Extract with a Quebracho (Schinopsis balansae Engl.) Extract on Methane Production and In vitro Rumen Fermentation Parameters. Animals 2022, 12, 1080. [Google Scholar] [CrossRef]
- Imani Moghadam, H.; Ghasemi, S.; Sobhanirad, S.; Behgar, M. Different levels of clove and savory essences on fermentative parameters in gas production technique and their antimicrobial effects on Peptostreptococcusanaerobius bacteria isolated from rumen. Iran. J. Anim. Sci. Res. 2021, 13, 335–349. [Google Scholar] [CrossRef]
- Kahvand, M.; Malecky, M. Dose-response effects of sage (Salvia officinalis) and yarrow (Achillea millefolium) essential oils on rumen fermentation in vitro. Ann. Anim. Sci. 2018, 18, 125–142. [Google Scholar] [CrossRef]
- Garcia, F.; Colombatto, D.; Brunetti, M.A.; Martínez, M.J.; Moreno, M.V.; Scorcione Turcato, M.; Lucini, E.; Frossasco, G.; Martínez Ferrer, J. The Reduction of Methane Production in the In vitro Ruminal Fermentation of Different Substrates is Linked with the Chemical Composition of the Essential Oil. Animals 2020, 10, 786. [Google Scholar] [CrossRef] [PubMed]
- Benchaar, C.; Greathead, H. Essential oils and opportunities to mitigate enteric methane emissions from ruminants. Anim. Feed Sci. Technol. 2011, 166–167, 338–355. [Google Scholar] [CrossRef]
- Velickovic, D.; Randjelovic, N.; Ristic, M.; Velickovic, A.N.A.; Smelcerovic, A. Chemical constituents and antimicrobial activity of the ethanol extracts obtained from the flower, leaf and stem of Salvia officinalis L. J. Serbian Chem. Soc. 2003, 68, 17–24. [Google Scholar] [CrossRef]
- Joch, M.; Cermak, L.; Hakl, J.; Hucko, B.; Duskova, D.; Marounek, M. In vitro Screening of Essential Oil Active Compounds for Manipulation of Rumen Fermentation and Methane Mitigation. Asian Australas. J. Anim. Sci. 2016, 29, 952–959. [Google Scholar] [CrossRef] [PubMed]
- Joch, M.; Mrázek, J.; Skřivanová, E.; Čermák, L.; Marounek, M. Effects of pure plant secondary metabolites on methane production, rumen fermentation and rumen bacteria populations in vitro. J. Anim. Physiol. Anim. Nutr. 2018, 102, 869–881. [Google Scholar] [CrossRef]
- Benchaar, C.; Chaves, A.V.; Fraser, G.R.; Beauchemin, K.A.; McAllister, T.A. Effects of essential oils and their components on in vitro rumen microbial fermentation. Can. J. Anim. Sci. 2007, 87, 413–419. [Google Scholar] [CrossRef]
- Króliczewska, B.; Pecka-Kiełb, E.; Bujok, J. Strategies Used to Reduce Methane Emissions from Ruminants: Controversies and Issues. Agriculture 2023, 13, 602. [Google Scholar] [CrossRef]
- Zmora, P.; Cieślak, A.; Pers-Kamczyc, E.; Szyszka, P.; Szumacher-Strabel, M. An in vitro study on the effect of sage, Salvia officinalis L., on rumen fermentation. J. Anim. Feed Sci. 2012, 21, 613–623. [Google Scholar] [CrossRef]
- Günal, M.; Pinski, B.; Abughazaleh, A.A. Evaluating the effects of essential oils on methane production and fermentation under in vitro conditions. Ital. J. Anim. Sci. 2017, 16, 500–506. [Google Scholar] [CrossRef]
- Patra, A.K.; Saxena, J. A new perspective on the use of plant secondary metabolites to inhibit methanogenesis in the rumen. Phytochemistry 2010, 71, 1198–1222. [Google Scholar] [CrossRef]
- Kurniawati, A.; Muhsin Al Anas, M. The effect of a candidate feed additive derived from the essential oils of Pinus merkusii (jungh. & de vriese) and Melaleuca leucadendra (L.) on the kinetics of gas production and methane emitted during in-vitro ruminal fermentation. BIO Web Conf. 2021, 33, 04009. [Google Scholar] [CrossRef]
- Kamra, D.N.; Pawar, M.; Singh, B. Effect of Plant Secondary Metabolites on Rumen Methanogens and Methane Emissions by Ruminants. In Dietary Phytochemicals and Microbes; Patra, A.K., Ed.; Springer: Dordrecht, The Netherlands, 2012; pp. 351–370. [Google Scholar]
- Kumar, S.; Dagar, S.S.; Sirohi, S.K.; Upadhyay, R.C.; Puniya, A.K. Microbial profiles, in vitro gas production and dry matter digestibility based on various ratios of roughage to concentrate. Ann. Microbiol. 2013, 63, 541–545. [Google Scholar] [CrossRef]
- Busquet, M.; Calsamiglia, S.; Ferret, A.; Kamel, C. Plant Extracts Affect In vitro Rumen Microbial Fermentation. J. Dairy Sci. 2006, 89, 761–771. [Google Scholar] [CrossRef]
- Mulyandari, F.; Yusiati, L.; Kurniawati, A. Parameter of ruminal feed fermentation in vitro with addition of clove essential oil (Syzygium aromaticum L.) as feed additive. In Proceedings of the IOP Conference Series: Earth and Environmental Science, 8th International Seminar on Tropical Animal Production, Yogyakarta, Indonesia, 23–25 September 2019; p. 012111. [Google Scholar]
- Kazana, P.; Siachos, N.; Panousis, N.; Petridou, A.; Mougios, V.; Valergakis, G.E. Phenotypical Variation of Ruminal Volatile Fatty Acids and pH during the Peri-Weaning Period in Holstein Calves and Factors Affecting Them. Animals 2022, 12, 894. [Google Scholar] [CrossRef]
- Hailemariam, S.; Zhao, S.; He, Y.; Wang, J. Urea transport and hydrolysis in the rumen: A review. Anim. Nutr. 2021, 7, 989–996. [Google Scholar] [CrossRef]
- Patra, A.K.; Yu, Z. Effects of vanillin, quillaja saponin, and essential oils on in vitro fermentation and protein-degrading microorganisms of the rumen. Appl. Microbiol. Biotechnol. 2014, 98, 897–905. [Google Scholar] [CrossRef]
- Benchaar, C.; Calsamiglia, S.; Chaves, A.V.; Fraser, G.R.; Colombatto, D.; McAllister, T.A.; Beauchemin, K.A. A review of plant-derived essential oils in ruminant nutrition and production. Anim. Feed Sci. Technol. 2008, 145, 209–228. [Google Scholar] [CrossRef]
- Guo, G.; Shen, C.; Liu, Q.; Zhang, S.-L.; Shao, T.; Wang, C.; Wang, Y.-X.; Xu, Q.-F.; Huo, W.-J. The effect of lactic acid bacteria inoculums on in vitro rumen fermentation, methane production, ruminal cellulolytic bacteria populations and cellulase activities of corn stover silage. J. Integr. Agric. 2020, 19, 838–847. [Google Scholar] [CrossRef]
- Fouts, J.Q.; Honan, M.C.; Roque, B.M.; Tricarico, J.M.; Kebreab, E. Enteric methane mitigation interventions. Transl. Anim. Sci. 2022, 6, txac041. [Google Scholar] [CrossRef]
- Roy, D.; Tomar, S.; Kumar, V. Rumen modulatory effect of thyme, clove and peppermint oils in vitro using buffalo rumen liquor. Vet. World 2015, 8, 203. [Google Scholar] [CrossRef]
- Carneiro, M.M.Y.; Goes, R.H.D.T.E.B.D.; Barros, B.C.B.; Oliveira, R.T.D.; Fernandes, A.R.M.; Silva, N.G.D.; Anschau, D.G.; Cardoso, C.A.L.; Oliveira, S.S.; Picanço, Y.D.S. Fatty acids profile, atherogenic and thrombogenic health lipid indices in the meat of lambs that received canola grain. Braz. J. Vet. Res. Anim. Sci. 2021, 58, e178023. [Google Scholar] [CrossRef]
- Al-Amiri, H.A.; Ahmed, N.; Al-Sharrah, T. Fatty acid profiles, cholesterol composition, and nutritional quality indices of 37 commonly consumed local foods in Kuwait in relation to cardiovascular health. medRxiv 2020. preprint. [Google Scholar] [CrossRef]
- Praagman, J.; Vissers, L.E.T.; Mulligan, A.A.; Laursen, A.S.D.; Beulens, J.W.J.; van der Schouw, Y.T.; Wareham, N.J.; Hansen, C.P.; Khaw, K.T.; Jakobsen, M.U.; et al. Consumption of individual saturated fatty acids and the risk of myocardial infarction in a UK and a Danish cohort. Int. J. Cardiol. 2019, 279, 18–26. [Google Scholar] [CrossRef]
- Carriquiry, M.; Weber, W.J.; Baumgard, L.H.; Crooker, B.A. In vitro biohydrogenation of four dietary fats. Anim. Feed Sci. Technol. 2008, 141, 339–355. [Google Scholar] [CrossRef]
- Mosley, E.E.; Powell, G.L.; Riley, M.B.; Jenkins, T.C. Microbial biohydrogenation of oleic acid to trans isomers in vitro. J. Lipid Res. 2002, 43, 290–296. [Google Scholar] [CrossRef]
- Proell, J.M.; Mosley, E.E.; Powell, G.L.; Jenkins, T.C. Isomerization of stable isotopically labeled elaidic acid to cis and trans monoenes by ruminal microbes. J. Lipid Res. 2002, 43, 2072–2076. [Google Scholar] [CrossRef]
- Ishlak, A.; Günal, M.; Abughazaleh, A.A. The effects of cinnamaldehyde, monensin and quebracho condensed tannin on rumen fermentation, biohydrogenation and bacteria in continuous culture system. Anim. Feed Sci. Technol. 2015, 207, 31–40. [Google Scholar] [CrossRef]
- Carreño, D.; Hervás, G.; Toral, P.G.; Belenguer, A.; Frutos, P. Ability of different types and doses of tannin extracts to modulate in vitro ruminal biohydrogenation in sheep. Anim. Feed Sci. Technol. 2015, 202, 42–51. [Google Scholar] [CrossRef]
- Shingfield, K.J.; Ahvenjärvi, S.; Toivonen, V.; Ärölä, A.; Nurmela, K.V.V.; Huhtanen, P.; Griinari, J.M. Effect of dietary fish oil on biohydrogenation of fatty acids and milk fatty acid content in cows. Anim. Sci. 2003, 77, 165–179. [Google Scholar] [CrossRef]
- Shingfield, K.J.; Kairenius, P.; Arölä, A.; Paillard, D.; Muetzel, S.; Ahvenjärvi, S.; Vanhatalo, A.; Huhtanen, P.; Toivonen, V.; Griinari, J.M.; et al. Dietary fish oil supplements modify ruminal biohydrogenation, alter the flow of fatty acids at the omasum, and induce changes in the ruminal Butyrivibrio population in lactating cows. J. Nutr. 2012, 142, 1437–1448. [Google Scholar] [CrossRef] [PubMed]
- Loor, J.J.; Doreau, M.; Chardigny, J.M.; Ollier, A.; Sebedio, J.L.; Chilliard, Y. Effects of ruminal or duodenal supply of fish oil on milk fat secretion and profiles of trans-fatty acids and conjugated linoleic acid isomers in dairy cows fed maize silage. Anim. Feed Sci. Technol. 2005, 119, 227–246. [Google Scholar] [CrossRef]
- Toral, P.G.; Hervás, G.; Leskinen, H.; Shingfield, K.J.; Frutos, P. In vitro ruminal biohydrogenation of eicosapentaenoic (EPA), docosapentaenoic (DPA), and docosahexaenoic acid (DHA) in cows and ewes: Intermediate metabolites and pathways. J. Dairy Sci. 2018, 101, 6109–6121. [Google Scholar] [CrossRef]
- Chow, T.T.; Fievez, V.; Moloney, A.P.; Raes, K.; Demeyer, D.; Smet, S.D. Effect of fish oil on in vitro rumen lipolysis, apparent biohydrogenation of linoleic and linolenic acid and accumulation of biohydrogenation intermediates. Anim. Feed Sci. Technol. 2004, 117, 1–12. [Google Scholar] [CrossRef]
- Micha, R.; Mozaffarian, D. Saturated Fat and Cardiometabolic Risk Factors, Coronary Heart Disease, Stroke, and Diabetes: A Fresh Look at the Evidence. Lipids 2010, 45, 893–905. [Google Scholar] [CrossRef]
- Mozaffarian, D.; Micha, R.; Wallace, S. Effects on Coronary Heart Disease of Increasing Polyunsaturated Fat in Place of Saturated Fat: A Systematic Review and Meta-Analysis of Randomized Controlled Trials. PLoS Med. 2010, 7, e1000252. [Google Scholar] [CrossRef]
- Pigsborg, K.; Gürdeniz, G.; Rangel-Huerta, O.D.; Holven, K.B.; Dragsted, L.O.; Ulven, S.M. Effects of changing from a diet with saturated fat to a diet with n-6 polyunsaturated fat on the serum metabolome in relation to cardiovascular disease risk factors. Eur. J. Nutr. 2022, 61, 2079–2089. [Google Scholar] [CrossRef]
Disclaimer/Publisher’s Note: The statements, opinions and data contained in all publications are solely those of the individual author(s) and contributor(s) and not of MDPI and/or the editor(s). MDPI and/or the editor(s) disclaim responsibility for any injury to people or property resulting from any ideas, methods, instructions or products referred to in the content.
|
Share and Cite
Bokharaeian, M.; Ghoorchi, T.; Toghdory, A.; Esfahani, I.J. The Dose-Dependent Role of Sage, Clove, and Pine Essential Oils in Modulating Ruminal Fermentation and Biohydrogenation of Polyunsaturated Fatty Acids: A Promising Strategy to Reduce Methane Emissions and Enhance the Nutritional Profile of Ruminant Products. Appl. Sci. 2023, 13, 11605. https://doi.org/10.3390/app132011605
Bokharaeian M, Ghoorchi T, Toghdory A, Esfahani IJ. The Dose-Dependent Role of Sage, Clove, and Pine Essential Oils in Modulating Ruminal Fermentation and Biohydrogenation of Polyunsaturated Fatty Acids: A Promising Strategy to Reduce Methane Emissions and Enhance the Nutritional Profile of Ruminant Products. Applied Sciences. 2023; 13(20):11605. https://doi.org/10.3390/app132011605Chicago/Turabian Style
Bokharaeian, Mostafa, Taghi Ghoorchi, Abdolhakim Toghdory, and Iman Janghorban Esfahani. 2023. “The Dose-Dependent Role of Sage, Clove, and Pine Essential Oils in Modulating Ruminal Fermentation and Biohydrogenation of Polyunsaturated Fatty Acids: A Promising Strategy to Reduce Methane Emissions and Enhance the Nutritional Profile of Ruminant Products” Applied Sciences 13, no. 20: 11605. https://doi.org/10.3390/app132011605